Digital Microfluidics (DMF) and Surface Plasmon Resonance (SPR) Biosensors for Assessing Anabolic and Catabolic Biomarkers in OA:
Digital microfluidic (DMF) platforms provide a scalable, reconfigurable and complete workflow for processing nL-range samples [1, 2]. The technology is based on droplets, which can be manipulated based on electrowetting (Figure 1).
TUE has sample experience on designing and fabricating these platforms at the NanoLabNL facility. We will integrate an optical sensor on the platform. The optical sensor is composed of microfabricated grating attached on top of a fiber tip where the light comes. The grating, composed of Si3N4 on a SiO2 substrate, will be designed to achieve a resonance wavelength in the range of 1000-1600 nm to be able to perform sensing at down to picomolar level [3] Finite Element Method simulations will be conducted to optimize the grating’s spectral response and surface sensitivity by varying geometric parameters. Next, the fabricated grating will be integrated into an experimental setup where reflectance spectra will be simultaneously measured using an optical spectrum analyzer and a fully integrated spectral interrogator. The interrogator features an array of tunable resonant-enhanced-cavity detectors on a chip operating in the near-infrared region. We will functionalize the surface of the DMF chip with antibodies that are designed specifically to bind pro- and anti-inflammatory markers with 1 x 1 mm2 area dedicated to each marker. The antibodies will be used because DMF is based on the application of electric fields and this might affect the molecular orientation of the aptamers with charged nature, decreasing the sensitivity limits. The markers involve IL-1ra, IL-4, IL-8, IL-10, IL-13, sTNF-R II, INF-γ, CXCL-1, CXCL7, CXCL12, CCL5, CD40L, SDF-1, HGF, IGF, VEGF and ENA-78 [4]. The method features the minimal processing volumes thanks to digital microfluidics integration while refractive index shifts registered by the fiber tip grating sensor will ensure low limit of detection from biological samples such as synovial fluid and blood serum. Integrating multi-omics data with biomarker readouts will be managed by employing ML algorithms.
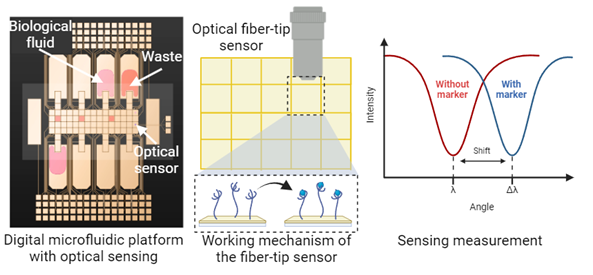
Figure 1. Digital microfluidics platform is composed of electrode plates (yellow tiles) that are used for manipulating droplets of different biological samples. The platform contains an integrated optical sensor that is selective for OA pro- and anti-inflammatory markers thanks to the antibody-functionalized surface.
The core concept revolves around utilizing microfluidics to develop a highly sensitive biosensor platform. A microfluidic chip featuring integrated gold surfaces functionalized with capture molecules for multiple OA biomarkers will serve as the model (Figure 2). The microfabrication process is assumed to yield consistent microchannel dimensions, ensuring uniform fluid dynamics and optimal biomarker interaction. The SPR technology is employed for real-time, label-free detection of biomolecular interactions. The integration of these SPR sensors within the microfluidic channels enables continuous, label-free monitoring of multiple biomarkers simultaneously, providing a powerful platform for early detection and monitoring of OA. Multi-omics approaches help integration of comprehensive biomarkers for OA to the system. Ensuring uniform microchannel dimensions and gold surface quality poses a significant challenge in the microfabrication process. This will be addressed by utilizing the advanced clean room facilities at the METU MEMS Center. Achieving high sensitivity and specificity in biomarker detection is another challenge, which will be met by optimizing the functionalization process of gold surfaces and validating the system. Integrating multi-omics data with SPR sensor outputs for comprehensive analysis presents a complexity that will be managed by employing ML algorithms. The primary anabolic markers will be Procollagen Type II C-Terminal Pro-peptide (PIICP) and Cartilage Oligomeric Matrix Protein (COMP) and the primary catabolic markers will be C-Terminal Telopeptide of Type II Collagen (CTX-II) and N-Terminal Telopeptide of Type I Collagen (NTX-I). The normal levels of these markers and their levels in patients with OA are given in Table. The selected biomarkers will be initially investigated following the fabrication of the microfluidic SPR platform. The platform will subsequently be refined and expanded to facilitate the detection of additional anabolic and catabolic biomarkers, informed by comprehensive multi-omics data integration.
Table. The normal vs patient levels of selected primary anabolic and catabolic OA markers.
Normal Levels | In OA Patients | |
PIICP | 50-250 ng/mL | – |
COMP | 0.5-1.5 µg/mL | 1.5-3 µg/mL or >3 µg/mL |
CTX-II | 200 pg/mL | 200-500 pg/mL or >500 pg/mL |
NTX-I | 5-25 nM | 25-40 nM or >40 nM |
Aptamers will be utilized as recognition layers within the microfluidic SPR sensor, which are short, single-stranded nucleic acids, offering several advantages over traditional recognition elements like antibodies. They are highly specific, stable and cost-effective, with the ability to be chemically synthesized and easily modified. The binding between an aptamer and its target molecule occurs through a combination of hydrogen bonding, van der Waals forces, and electrostatic interactions, enabling the aptamer to fold into unique three-dimensional structures that can selectively and tightly bind to the target. Utilizing aptamers in place of antibodies significantly enhances the shelf-life of the sensor platform, offering a crucial advantage in the stability and longevity of the detection system. The fabrication of the µOsteoGuard Multi as well as the immobilization technique for the aptamers are explained detailly in WP7 description table.
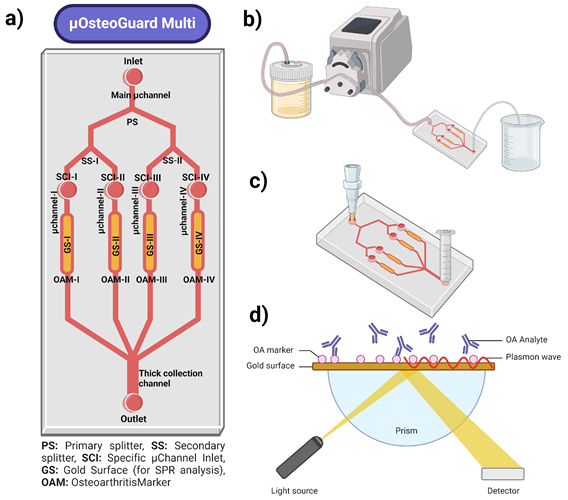
Figure 2. a) Schematic presentation of the microfluidic SPR sensor with four independent detection zones to provide simultaneous read-out for different OA markers, b) peristaltic pump system for the surface modification steps, c) tubing-free state of the microfluidic sensor where sample flow will be provided via a tilted platform, d) basics of the SPR sensing.
References:
[1] Mok, J., Mindrinos, M. N., Davis, R. W., & Javanmard, M. (2014). Digital microfluidic assay for protein detection. Proceedings of the National Academy of Sciences, 111(6), 2110-2115.
[2] Ng, A. H., Fobel, R., Fobel, C., Lamanna, J., Rackus, D. G., Summers, A., … & Wheeler, A. R. (2018). A digital microfluidic system for serological immunoassays in remote settings. Science translational medicine, 10(438), eaar6076
[3] Picelli, L., van Klinken, A., Lindgren, G., Hakkel, K. D., Pagliano, F., Fiaschi, N., … & Fiore, A. (2020). Scalable wafer-to-fiber transfer method for lab-on-fiber sensing. Applied Physics Letters, 117(15).